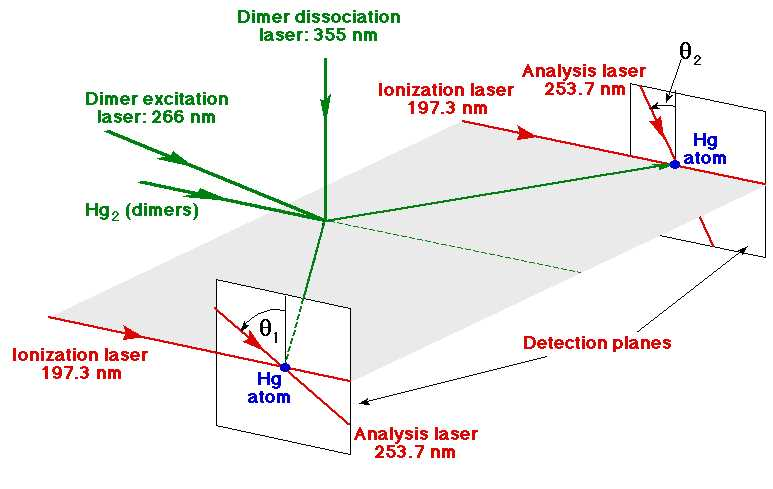
This project is performed in collaboration with Dr. Fry (original concept). It will be the first test of the Bell inequalities based on atoms and is a direct realization of Bohm's version of the Einstein-Podolsky-Rosen. Since atoms move much more slowly than photons, the lifetime of the entangled state will be much longer, several orders of magnitude longer than in previous experiments. Due to its unique features, the experiment will close the previously mentioned loopholes associated with all previous tests of the Bell inequalities. Besides its important fundamental aspects the experiment offers some interesting technical challenges as well.
The beginning of this century marked a revolution in Physics. In merely 30 years, starting with Planck's explanation of the black body radiation in 1900, the picture of physics was radically changed by the introduction of Quantum Mechanics. Quantum Mechanics is a very counterintuitive theory that continues to puzzle physicists throughout the world. Its interpretation is still debated, new ways of interpretation are introduced almost daily.
Ironically, Einstein was one of the main players in the introduction of Quantum Mechanics. Specifically, he explained the photo effect with Planck's energy quanta, which were later called photons and introduced the concepts of absorption and spontaneous and stimulated emission of radiation. Despite these tremendeous advances Einstein contributed to making Quantum Mechanics possible, Einstein always doubted the completeness of Quantum Mechanics. Specifically, the random nature of Quantum Mechanics and the spooky action-at-a-distance (non-locality) were things he deeply rejected. His famous quote “Subtle is the Lord, but he does not play dice!” illustrates his attitude towards Quantum Mechanics.
In 1935, together with Rosen and Podolsky, he published his famous article “Can Quantum Mechanical Description be considered complete?” (Einstein, 1935). In this paper, he introduces a gedankenexperiment (EPR-Experiment), illustrating the deficencies of Quantum Mechanics.
In the following I will briefly sketch the experiment. I will, however, use Bohm's version of the experiment introduced in the 1950's. Conceptually, it is easier to understand than Einstein's original gedankenexperiment. Suppose you have a system consisting of two particles, each with spin 1/2 in an entangled state, i.e
|?>=(|+>1|?>2 ? |?>1|+>2),
where the + and – indicate spin up and down, respectivley. The indices indicate the particle number. The system is therefore in a state with a total spin S=0, i.e. the singlet state. If particle one is found with spin up (+), particle two has to be in spin down (-) and vice versa. Please note, however, that the direction with respect to which the spins are oriented is not defined. Only the relative spin orientation of the particles is known. This is one of the things that Einstein disliked. He felt that the particles should have some kind of tag (hidden variables) associated with them, that would predetermine the orientation. The gedankenexperiment now involves the separation of the two particles and the independent measurement of their spin orientation. The entanglement between the two particles produces the non-local character or action-at-a-distance. In principle one could perform the measurement for one particle here on earth, while the second particle travels light years away. And yet quantum mechanics tells me that immediately when spin up for one particle is measured, the other one has to be down. This collapse of the wave function occurs instantaneously without the need of some signal transfer limited by the speed of light. Finally, Einstein came to the conclusion that there is a contradiction in the experiment with the quantum mechanical concept of commuting operators. The spin (up/down) can be measured on particle one. Consequently the spin direction of particle 2 is inferred from the result of this measurement. By contrast, a measurement of spin left/right can be performed on that very particle. Seemingly, you have information of spin up/down and left/right for one particle producing a contradiction of quantum mechanics.
For a long time no progress in this problem was made. Arguments were only philosophical in nature until 1964 when Bell proved that local hidden variable (LHV) theories that complete Quantum Mechanics in the Einsteinian sense could exist. Moreover, he showed that the statistical predictions of correlations in an EPR type experiment by any LHV theory obey an inequality whereas the corresponding predictions of Quantum Mechanics can violate this inequality. In other words, due to their local character correlations LHV theories show weaker than in Quantum mechanics. For the first time experiments that were able to decide between LHV theories and Quantum Mechanics were possible.
Experimental requirements for these tests are high: high detection efficiency, strong correlation, i.e. a very pure entangled state, very good analyzers and finally a high spatial correlation of the two particles. As a result, it took 8 years for the first tests to be performed. And still today the final answer has not been determined due to the existing loopholes in all previous experiments.
I will focus in this overview on recent tests of the Bell inequalities that were mostly based on entangled states between photons. For a more detailed review, see this paper.
In the first experiments, the entangled states were produced via cascade decays of atoms, where the selection rules for dipole allowed transitions lead to an entanglement of the polarization between the two emitted photons. The earliest tests took place in 1972 and were performed by Clauser and coworkers (Clauser, 1972). Later Holt, Clauser and Fry performed additional experiments, the latter of which was the first one based on lasers. In the early 80's these experiments were improved by Aspect and coworkers (Aspect, 1980). They made the first attempt to enforce the locality condition by switching between different analyzers.
More recent experiments produced entanglement through parametric down conversion: a UV photon is split by the non-linear interaction within a crystal into two IR photons. Those experiments were pioneered by Shih (Shih, 1987) and Mandel et al. (Mandel, 1988) in so called type-I sources and developed further by Kwiat, Shih and Zeilinger et al. (Kwiat, 1995). The important step in the latter experiments was to switch to parametric down conversion sources of type-II. In those the entangled pairs are directly produced, resulting in large count rates and hence good signal/noise ratios in the experiment. This is important to achieve good statistics and hence meaningful results. Recently, Gisin and coworkers (opens in new tab) have experimentally demonstrated that the entanglement between two photons exists over a long time (Gisin, 1998). (Some scientists have explained the results of the photon experiments by the short time scales involved.) They performed a test of the Bell inequalities over a large distance with optical fibers. Unfortunately, due to their specific setup the detection efficiency loophole could not be closed in these experiments. However, they represent an important step foward.
In the latest development in the series of these experiments, Zeilinger and his group enforced the locality conditions by separating the two analyzers by approximately 400 m and randomly switching the orientation of the analyzing polarizers (Zeilinger, 1998). The year 2000 marked another important step forward. Wineland and coworkers performed a Bell inequalities test based on the entanglement of ions in traps, where they achieved a very high detection efficiency. Their detection scheme, however, does not allow them to enforce the locality condition simultaneously (Wineland, 2001).
In summary, tremendous progress has been made in the last couple of years from the conception of the Bell inequalities to the latest tests. Most of the experiments gave agreement with Quantum Mechanics. No experiment to date closes all loopholes simultaneously. A rigorous test of the Bell inequalities has not been performed.
The strongest form of the Bell inequalities – the Bell-Clauser-Horne inequality – involves the measurement of coincidence and singles rates of spin components. Specifically, it involves the measurement of R++(Θ1,Θ2), which is the probability of finding particle 1 in spin up with respect to angle Θ1 and particle 2 in spin up with respect to angle Θ2. This measurement is performed for 4 sets of angles, two for each detector including the four possible combinations. Furthermore the single rate R1+(Θ1) must be measured. The Bell inequality is then:
S(Θ1,Θ'1,Θ2,Θ'2)=(R++(Θ'1,Θ'2) -R++(Θ1,Θ2) +R++(Θ'1,Θ2) +R++(Θ1,Θ'2))/ (R1+(Θ1)+ R2+(Θ2))<=1
The quantum mechanical prediction for these coincidence and singles rates can be calculated and plugged into the equation. For the case of maximum violation of Quantum Mechanics we find
S(135o,225o,0o,90o)=1/2 ηgε+(1+sqrt(2) ε2-/ε2+ ),
where η is the detection efficiency, g is the conditional probability of finding particle 2 in detector one provided that particle 1 entered in detector 1 and ε+ and ε- are measures of the quality of the analyzers. It is clear that quantum mechanics only violates this inequality if the above mentioned parameters are as close as possible to unity. Only in this case can a test of the inequality be performed.
In all previous experiments, however, this was not the case.
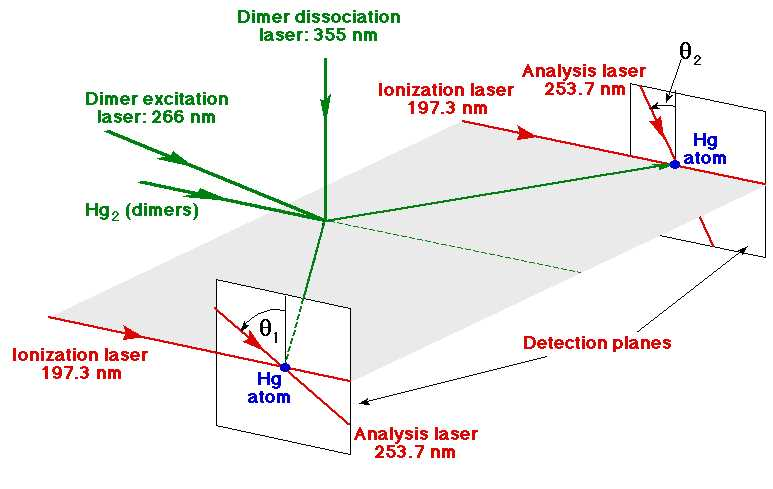
Rather than being based on the polarization correlation of photons it is based on entangled states between atoms. The entangled singlet state of two 199Hg atoms, each with nuclear spin 1/2, is produced by two-photon induced dissociation of the mercury dimer, 199Hg2. The measurement of the spin correlations between the two spatially separated Hg atoms is based on the use of polarized laser beams, which selectively ionize only one spin state.
199Hg was selected, since it has no angular momentum, no electron spin, but nuclear spin of F=1/2. This simplifies the analysis of the spin states. Furthermore, the nuclear spin is relatively immune to environmental conditions, i.e. magnetic fields etc., making it easier to preserve the entanglement. The Hg dimer is produced in a supersonic jet expansion. The preparation of the entangled state is based on a two-photon dissociation scheme. First, a laser pulse at 266 nm excites the dimers in an excited electronic state. A second laser pulse (355 nm) pumps them back down onto a dissociative part of the potential energy surface. The dimer falls apart and the two fragments start moving towards their respective detectors. The ability to only dissociate dimers in a singlet state is based on the symmetry properties of the molecular wave function. Briefly, the two Hg atoms are Fermions. Hence the overall wavefunction has to conform to the Pauli exclusion principle. Considering the symmetries of the different parts of the overall wavefunction (electronic, vibrational, rotational and nuclear (singlet wavefunction)), the excitation has to be restricted to dimers in even rotational state. This can be achieved through spectroscopic selection.

After their dissociation the two particles move towards their respective detectors in which they are analyzed for their spin direction. Since the nuclear moment is too weak, a Stern-Gerlach analyzer cannot be used for the analysis. Instead, we use a two photon ionization step that makes use of the selection rules for Zeeman sublevels.
Specifically, the Hg atom has nuclear spin 1/2, i.e. two Zeeman sublevels m=-1/2 and m=+1/2 corresponding to spin up and down, respectively. Suppose the first laser is left hand circular polarized. Therefore we require for dipole allowed transitions, Deltam=-1. Hence, a Hg atom in state m=1/2 will be excited into the m=-1/2 state of level 2 (see fig. below). A particle in the m=-1/2 state will not be excited, since their is no appropriate level in the intermediate state. In the second step the ionizing laser takes the atom to an autoionizing state, where it decays into a positively charged ion and an electron. Simulations show that the efficiency achievable is larger than 99%! Atoms in the m=-1/2 state can only be ionized through a much less efficient route, i.e. the F=3/2 component of the 6s6p state. It is detuned by 22 GHz. Requirement of the efficiency is a very well defined Opens internal link in current windowtiming of the two lasers involved. The quantization axis, with respect to which the spin analysis is conducted, is represented by the propagation direction of the first circular polarized laser beam (only in that direction, the polarization is circular.) The experiment therefore involves rotation of the 253.7 nm laser beams at the two detectors.
The experiment requires four lasers in the UV spectral range. Specifically, we need the laser radiation at the wavelength listed in the table. Along with the wavelength, you also find the type of laser used as well as some details on the specs of the systems.
The most stringent requirements have to be fulfilled by the Alexandrite and the Ti:Sapphire laser systems.
The Alexandrite laser is used to spectroscopically select only dimer within a very narrow velocity window. This increases the propability of finding both Hg atoms of one pair in the detectors. Therefore, the Alexandrite laser has to have a very narrow frequency distribution, which requires long pulses. We opted for the Alexandrite laser since it shows long laser pulses. We have successfully reduced the linewidth of our laser to < 8 MHz..
A highly efficient spin analysis is necessary in order to close the loopholes associated with previous experiments. Simulations show that this is only possible if the two laser pulse arrive within a very narrow timing window, i.e. 2 nanoseconds with respect to each other. Therefore we decided to run a flashlamp pumped Ti:Sapphire laser simultaneously on two wavelengths. The exact timing is achieved using an optical delay line.
Laser necessary in this experiment
Wavelength | Usage | Type | Non-linear Conv. | Pulse length | Energy | Fund. Linewidth | Remark |
---|---|---|---|---|---|---|---|
266 nm | Excitation of Dimer | Alexandrite | Third-Harmonic | 120 ns | 1 mJ | 8 MHz | tunable, injection seeded |
355 nm | Dissociation of Dimer | Ti:Sapphire | Second-Harmonic | 8 ns | 1 mJ | 100 MHz | tunable. injection seeded |
253.7 nm | Spin Selection | Ti:Sapphire | Third-Harmonic | 8 ns | > 200 muJ | < 100 MHz | regenerative amplifier, timing critical |
197.3 nm | Photo-Ionization | Ti:Sapphire | Fourth-Harmonic | 8 ns | >60 muJ | < 100 MHz | regenerative amplifier, timing critical |
Here is a list of references for further reading on the subject:
- A. Einstein, B. Podolsky, N. Rosen; Phys. Rev. 47, 777, (1935)
- J. Bell; Physics 1, 195, (1964)
- S.J. Freedman, J.F. Clauser; Phys. Rev. Lett. 28 938, (1972)
- J.F. Clauser, A. Shimony; Rep. prog. Phys. 41, 1881, (1978)
- E.S. Fry, R.C. Thompson; Phys. Rev. Lett. 37, 465, (1976)
- A. Aspect, J. Dalibard, G. Roger; Phys. Rev. Lett. 49, 1804, (1982)
- C.O. Alley and Y. H. Shih, Proc. Int. Symp. Foundations of Quantum Mechanics, 47, 1987
- Z. Y. Ou, L. Mandel; Phys. Rev. Lett. 61, 50, (1988)
- P.G. Kwiat, K. Mattle, H. Weinfurter, A. Zeilinger, A.V. Sergienko, Y. Shih; Phys. Rev. Lett. 75, 4337, (1995)
- E.S. Fry, Th. Walther, S. Li; Phys. Rev. A 52, 4381, (1995)
- W. Tittel, J. Brendel, H. Zbinden, N. Gisin; Phys. Rev. Lett. 81, 3563, (1998)
- G. Weihs, Th. Jennewein, C. Simon, H. Weinfurter, A. Zeilinger; Phys. Rev. Lett. 81, 5039 (1998).
- E.S. Fry and Th. Walther, in Advances in atomic, molecular and optical Physics, Vol. 42, p.1, (Academic Press, New York), 2000.
- M.A Rowe, D. Kielpinski, V. Meyer, C.A. Sackett, W.M. Itano, C. Monroe, D.J. Wineland; Nature 409, 791-794 (2001)
- E.S. Fry, Th. Walther; in Quantum (Un)Speakables ed. by R.A. Bertlmann and A. Zeilinger, Springer (Heidelberg) 103, (2002)